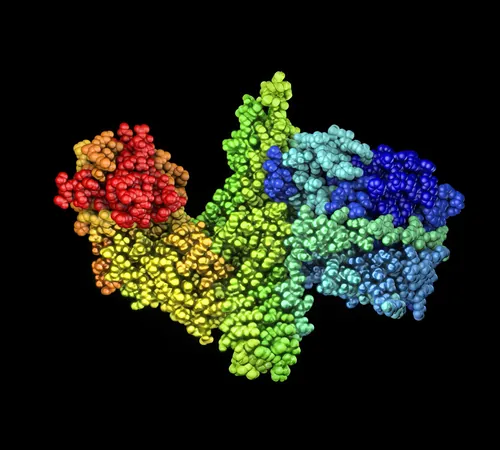
Breathing Without Oxygen: How Ancient Microbes Fuel Life Without Air
2025-03-21
Author: Siti
Introduction
Earth's current biosphere is rich with life forms that depend on oxygen for respiration, combining sugars with this essential gas to produce carbon dioxide and water, ultimately generating ATP – the vital energy currency that powers all biological processes.
However, billions of years ago, when Earth was still forming its atmosphere, oxygen was nowhere to be found. This raises an intriguing question: how did early organisms produce energy when oxygen was absent?
Unlocking the Secrets of Oxygen-Free Metabolism
Recent groundbreaking research sheds light on this mystery, focusing on primordial microbes that still thrive in modern environments devoid of oxygen, such as the deep-sea hot springs. These ancient organisms have survived through a unique form of respiration that combines carbon dioxide and hydrogen to create acetic acid, a process that ultimately results in ATP production.
Today, most life forms utilize oxygen-based respiration, but many ecosystems, including some extreme environments, continue to lack oxygen. These habitats are home to microorganisms that tap into alternative energy-producing pathways. One of these involves the reaction with CO₂ and hydrogen.
Understanding these ancient processes is not only crucial for piecing together how life functioned in the absence of oxygen billions of years ago but could also pave the way for new biotechnological advancements.
The research led by Professor Volker Müller at Goethe University Frankfurt illuminates the exact mechanisms by which these microbes generate ATP through acetic acid production, revealing a sophisticated energy system dependent on sodium ions.
The Ion-Powered Mechanism of Energy Production
Professor Müller’s findings highlight how the process of creating acetic acid actively triggers the expulsion of sodium ions from the bacterial cells into the surrounding environment. This activity reduces sodium concentration within the cell, and the cell membrane acts as a barrier, holding back this ion flow. When this barrier is breached, sodium ions rush back into the cell, driving ATP synthesis akin to a molecular turbine.
This system showcases a remarkable adaptation: rather than using oxygen for electron transport as many organisms do, these ancient microbes leverage sodium ion gradients to fuel their energy needs.
The Role of Proteins in Ancient Microbes
At the core of this energy production mechanism lies the Rnf complex, a protein assembly embedded in the cell membrane. This complex facilitates electron transfer from hydrogen to carbon dioxide in the formation of acetic acid, a process that researchers have only recently begun to fully describe due to the Rnf's delicate structure.
By employing cryo-electron microscopy, scientists managed to capture high-resolution images of the Rnf complex from *Acetobacterium woodii*, allowing them to construct detailed 3D models that provide insight into how these proteins interact to facilitate electron flow.
The Dynamics of Sodium Ion Pumping
Although the structure of the proteins was decoded, how electron movement correlates with sodium ion expulsion remained a challenge. A pivotal molecular dynamics simulation led by Professor Ville Kaila from Stockholm University unveiled that a crucial iron-sulfur cluster in the membrane was responsible for attracting sodium ions as it gained electrons, much like a magnet.
The subsequent interaction caused shifts in the structure of the proteins, creating openings for sodium ions to exit the cell. This efficient cycle allows sodium gradient accumulation, ultimately powering the ATP synthase that produces ATP.
Implications for the Future: Carbon Capture and Drug Development
This significant discovery extends beyond understanding ancient metabolism; it also harbors potential real-world applications, particularly in carbon capture technologies. By further unraveling these microbial processes, industries could utilize bacteria for greenhouse gas mitigation or to synthesize high-value chemicals.
Moreover, similar respiratory enzymes found in various pathogens raise the intriguing possibility of drug development targeting these proteins, providing new avenues for combating bacterial infections.
By illuminating how life persisted and adapted in conditions lacking oxygen, this study underscores the resilience and ingenuity of early life forms on Earth. Such discoveries not only deepen our understanding of life's evolution but also broaden the horizons for innovative solutions in biotechnology today.
This transformative research is documented in the journal *Nature Communications*, where it adds a new chapter to our ongoing exploration of life's complexities.